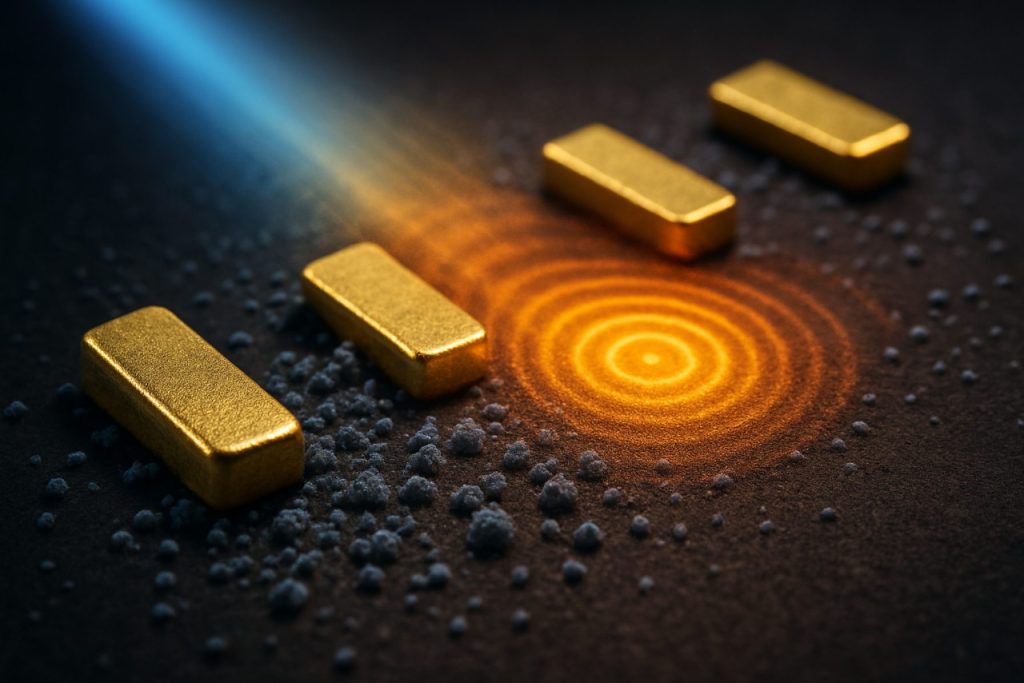
Fano Resonance in Plasmonic Nanostructures: The Quantum Interference Revolutionizing Light-Matter Interactions. Discover How Subtle Asymmetries Drive Breakthroughs in Sensing, Switching, and Beyond.
- Introduction to Fano Resonance: Historical Context and Fundamentals
- Plasmonic Nanostructures: Types, Fabrication, and Optical Properties
- Theoretical Framework: Quantum Interference and Asymmetric Line Shapes
- Experimental Observation of Fano Resonance in Plasmonic Systems
- Design Strategies for Engineering Fano Resonance
- Tunable Fano Resonances: External Stimuli and Dynamic Control
- Applications in Sensing: Ultra-Sensitive Detection and Biosensing
- Fano Resonance in Nonlinear and Active Plasmonic Devices
- Challenges and Limitations in Real-World Implementations
- Future Directions: Emerging Trends and Unexplored Opportunities
- Sources & References
Introduction to Fano Resonance: Historical Context and Fundamentals
Fano resonance is a fundamental phenomenon in wave physics, characterized by an asymmetric spectral line shape resulting from the interference between a discrete resonance and a broad spectral continuum. The concept was first introduced by Italian physicist Ugo Fano in 1961, who described the effect in the context of atomic photoionization, where the interaction between a bound state and a continuum of states leads to distinctive asymmetric profiles in absorption spectra. This theoretical framework has since been extended to a wide range of physical systems, including optics, condensed matter, and nanophotonics.
In the realm of plasmonics, Fano resonance has garnered significant attention due to its ability to enhance and control light-matter interactions at the nanoscale. Plasmonic nanostructures—typically composed of noble metals such as gold or silver—support collective oscillations of conduction electrons known as surface plasmons. When these nanostructures are engineered to support both a narrow discrete plasmonic mode and a broad continuum mode, their interaction can give rise to Fano resonances. The resulting spectral features are highly sensitive to the geometry, composition, and environment of the nanostructure, making them valuable for applications in sensing, switching, and nonlinear optics.
The fundamental mechanism underlying Fano resonance in plasmonic systems involves the constructive and destructive interference between the two types of modes. For example, in a typical plasmonic oligomer or a coupled nanoparticle system, a bright (superradiant) mode with a broad linewidth interacts with a dark (subradiant) mode with a narrow linewidth. The interference between these modes leads to a sharp asymmetric dip or peak in the extinction or scattering spectrum, which is the hallmark of Fano resonance. This effect can be precisely tuned by adjusting the structural parameters of the nanostructure, such as the size, shape, and arrangement of the constituent nanoparticles.
The study of Fano resonance in plasmonic nanostructures is supported by leading scientific organizations and research institutions worldwide, including Nature Publishing Group and American Physical Society, which regularly publish pioneering research in this field. The unique properties of Fano resonances—such as their sharp spectral features and high sensitivity—have positioned them at the forefront of nanophotonics research, with ongoing efforts to exploit these effects for advanced optical devices and biosensors.
Plasmonic Nanostructures: Types, Fabrication, and Optical Properties
Fano resonance is a distinctive optical phenomenon that arises from the interference between a broad spectral line (continuum) and a narrow discrete resonance. In the context of plasmonic nanostructures, Fano resonances manifest as asymmetric line shapes in the optical spectra, resulting from the coupling between bright (superradiant) and dark (subradiant) plasmonic modes. This effect is of significant interest due to its potential for enhancing light-matter interactions, enabling highly sensitive detection, and facilitating novel photonic devices.
Plasmonic nanostructures, typically composed of noble metals such as gold and silver, support localized surface plasmon resonances (LSPRs)—collective oscillations of conduction electrons at the metal-dielectric interface. When these nanostructures are engineered into complex geometries, such as oligomers, nanorings, or coupled nanoparticle arrays, they can support both bright and dark plasmonic modes. The interaction between these modes under external electromagnetic excitation leads to Fano resonance. For example, in a plasmonic “dolmen” structure (a configuration of three or more nanoparticles), the bright mode is directly excited by incident light, while the dark mode is only excited through near-field coupling, resulting in the characteristic asymmetric Fano profile.
The fabrication of plasmonic nanostructures capable of supporting Fano resonances requires precise control over geometry and spatial arrangement at the nanoscale. Techniques such as electron beam lithography, focused ion beam milling, and self-assembly are commonly employed to achieve the necessary structural fidelity. Advances in nanofabrication have enabled the realization of complex architectures, including asymmetric nanoparticle clusters and metamaterials, which are essential for tailoring the Fano resonance properties.
Optically, Fano resonances in plasmonic nanostructures are characterized by sharp spectral features and high sensitivity to changes in the local environment. This makes them particularly attractive for applications in biosensing, where the detection of minute refractive index changes is crucial. The tunability of Fano resonances—achieved by varying the size, shape, and arrangement of the constituent nanoparticles—further enhances their utility in designing responsive optical devices, such as switches, filters, and modulators.
Research into Fano resonances in plasmonic systems is supported by leading scientific organizations and research institutions worldwide, including Nature Publishing Group and American Physical Society, which regularly publish peer-reviewed studies on the topic. The continued development of advanced fabrication methods and theoretical models is expected to drive further innovations in the exploitation of Fano resonances for next-generation photonic and sensing technologies.
Theoretical Framework: Quantum Interference and Asymmetric Line Shapes
Fano resonance is a fundamental phenomenon in physics, characterized by an asymmetric spectral line shape arising from the quantum interference between a discrete resonance and a continuum of states. In the context of plasmonic nanostructures, this effect emerges when localized surface plasmon resonances (LSPRs)—collective oscillations of conduction electrons in metallic nanoparticles—interact with broad spectral backgrounds or other resonant modes. The resulting interference leads to sharp, asymmetric features in the optical response, which are highly sensitive to the nanostructure’s geometry, composition, and surrounding environment.
The theoretical framework for Fano resonance in plasmonic systems is rooted in the original work of Ugo Fano, who described the interaction between a discrete quantum state and a continuum, resulting in a characteristic line shape governed by the so-called Fano parameter (q). In plasmonic nanostructures, the discrete state is typically associated with a narrow plasmonic mode, while the continuum may arise from a broad dipolar resonance or the radiative background. The interference between these pathways modifies the absorption, scattering, or extinction spectra, producing a profile that deviates from the symmetric Lorentzian shape typical of isolated resonances.
Mathematically, the Fano line shape can be expressed as:
- F(ε) = (q + ε)2 / (1 + ε2),
where ε is the reduced energy (relative to the resonance), and q quantifies the degree of asymmetry. The value of q depends on the coupling strength and phase difference between the discrete and continuum channels. In plasmonic nanostructures, this coupling can be engineered by designing specific geometries—such as dolmen structures, nanorod dimers, or nanoparticle clusters—that support both narrow and broad plasmonic modes. The resulting Fano resonances are tunable through structural parameters, enabling precise control over the optical response.
The significance of Fano resonance in plasmonics extends beyond fundamental physics. The sharp spectral features and high sensitivity to environmental changes make Fano-resonant nanostructures promising for applications in biosensing, nonlinear optics, and light manipulation at the nanoscale. The ability to tailor quantum interference effects in engineered nanostructures is a key aspect of modern nanophotonics research, as recognized by leading scientific organizations such as the Optica (formerly Optical Society of America) and the American Physical Society, both of which actively support research in plasmonics and quantum optics.
Experimental Observation of Fano Resonance in Plasmonic Systems
The experimental observation of Fano resonance in plasmonic systems has been a pivotal development in nanophotonics, enabling the manipulation of light at the nanoscale with unprecedented precision. Fano resonance arises from the interference between a broad spectral line (continuum) and a narrow discrete resonance, resulting in an asymmetric line shape in the optical response. In plasmonic nanostructures, this phenomenon is typically realized by engineering the interaction between different plasmonic modes, such as bright (superradiant) and dark (subradiant) modes, within metallic nanostructures.
Early experimental demonstrations of Fano resonance in plasmonic systems utilized carefully designed metallic nanostructures, such as coupled nanorods, nanoshells, and nanoparticle clusters. For instance, asymmetric arrangements of gold or silver nanoparticles have been fabricated using electron-beam lithography and self-assembly techniques, allowing precise control over the geometry and coupling strength between the constituent elements. These structures support both dipolar (bright) and higher-order (dark) plasmonic modes, whose interaction gives rise to the characteristic Fano line shape in the extinction or scattering spectra.
A notable example is the use of dolmen-type and disk-ring nanostructures, where the symmetry breaking enables the coupling between bright and dark modes. Experimental measurements, often performed using dark-field optical microscopy and spectroscopy, reveal sharp asymmetric features in the optical spectra, directly evidencing Fano resonance. The tunability of the Fano resonance is achieved by varying structural parameters such as the gap distance, size, and arrangement of the nanoparticles, which in turn modulate the coupling strength and spectral position of the resonance.
The observation of Fano resonance in plasmonic systems has been corroborated by advanced characterization techniques, including near-field scanning optical microscopy (NSOM) and electron energy loss spectroscopy (EELS). These methods provide spatially resolved information about the electromagnetic field distribution and mode profiles, confirming the interference mechanism underlying the Fano effect. Furthermore, the experimental findings are often supported by numerical simulations based on finite-difference time-domain (FDTD) or finite element methods, which help interpret the observed spectral features and guide the design of new nanostructures.
The ability to experimentally realize and control Fano resonance in plasmonic nanostructures has significant implications for applications in sensing, switching, and nonlinear optics. The sharp spectral features and high sensitivity to environmental changes make these systems promising for label-free biosensing and active photonic devices. Research in this area is actively supported by leading scientific organizations such as the Nature Publishing Group and the Optica (formerly Optical Society of America), which regularly publish pioneering studies and reviews on the topic.
Design Strategies for Engineering Fano Resonance
The engineering of Fano resonance in plasmonic nanostructures relies on the deliberate design of nanoscale architectures that facilitate the interference between discrete and continuum plasmonic modes. Fano resonance, characterized by its asymmetric spectral line shape, arises when a narrow, discrete resonance (often a localized surface plasmon mode) interacts with a broad spectral continuum (such as a propagating plasmon or a radiative mode). The ability to tailor this interaction is central to achieving sharp spectral features and enhanced sensitivity in applications ranging from biosensing to nonlinear optics.
A primary design strategy involves the use of asymmetric nanostructures. By breaking the symmetry of plasmonic elements—such as in dolmen-type, split-ring, or disk-ring configurations—engineers can induce coupling between bright (radiative) and dark (subradiant) modes. For example, in a dolmen structure, a central bar (bright mode) is flanked by parallel side bars (dark modes), and their near-field coupling produces the characteristic Fano profile. The geometric parameters, including gap size, orientation, and relative positioning, are critical in tuning the resonance energy and the degree of asymmetry in the spectral response.
Another approach leverages plasmonic oligomers, which are clusters of metallic nanoparticles arranged to support collective modes. In these systems, Fano resonances emerge from the interference between the superradiant (symmetric) and subradiant (asymmetric) collective oscillations of the nanoparticles. The precise control of interparticle spacing and arrangement enables the modulation of the Fano resonance’s spectral position and linewidth.
Hybrid nanostructures—combining plasmonic materials with dielectric or semiconductor components—offer further flexibility. For instance, integrating a metallic nanodisk with a dielectric ring can support both plasmonic and Mie resonances, whose interaction can be engineered to produce Fano-like features. This hybridization allows for the exploitation of low-loss dielectric modes to sharpen the resonance and reduce dissipative losses inherent to metals.
Advanced fabrication techniques, such as electron-beam lithography and focused ion beam milling, are essential for realizing these complex nanostructures with the required precision. Computational electromagnetic simulations, including finite-difference time-domain (FDTD) and finite element methods (FEM), are routinely employed to predict and optimize the Fano resonance characteristics before experimental realization.
The design of Fano-resonant plasmonic nanostructures is a multidisciplinary effort, drawing on advances in nanofabrication, materials science, and theoretical modeling. Research in this area is supported by leading scientific organizations such as the Nature Publishing Group and the Optica (formerly Optical Society of America), which regularly publish seminal work on the topic. These strategies collectively enable the precise engineering of Fano resonances, paving the way for next-generation photonic devices with enhanced performance and functionality.
Tunable Fano Resonances: External Stimuli and Dynamic Control
Fano resonances in plasmonic nanostructures are characterized by their distinctive asymmetric spectral line shapes, arising from the interference between a broad (continuum) plasmonic mode and a narrow (discrete) resonance. A key advantage of these resonances is their tunability, which enables dynamic control over optical properties for applications in sensing, switching, and modulation. The ability to actively tune Fano resonances is crucial for the development of next-generation photonic devices.
External stimuli provide versatile mechanisms for modulating Fano resonances in plasmonic nanostructures. Among the most widely explored stimuli are electrical gating, optical pumping, thermal effects, and mechanical deformation. Each approach leverages the sensitivity of plasmonic modes to changes in the local dielectric environment, carrier density, or structural configuration.
Electrical control is often achieved by integrating plasmonic nanostructures with materials whose carrier concentration can be modulated, such as graphene or semiconductor substrates. By applying a gate voltage, the refractive index near the nanostructure is altered, shifting the Fano resonance position and modifying its spectral profile. This method offers fast and reversible tuning, making it attractive for active plasmonic devices. Research institutions such as Massachusetts Institute of Technology and Stanford University have demonstrated electrically tunable Fano resonances in hybrid plasmonic-graphene systems.
Optical tuning involves the use of intense light pulses to induce transient changes in the electronic or lattice structure of the plasmonic material or its environment. For example, photoexcitation can generate hot carriers or modify the refractive index, leading to ultrafast modulation of the Fano resonance. This approach enables sub-picosecond switching speeds, as shown in studies by groups at California Institute of Technology and University of Cambridge.
Thermal tuning exploits the temperature dependence of material properties. By locally heating the nanostructure or its substrate, the dielectric function can be modified, resulting in a shift of the Fano resonance. This method is particularly relevant for sensors operating in variable thermal environments.
Mechanical tuning, such as stretching or compressing flexible substrates with embedded plasmonic nanostructures, can dynamically alter the geometry and coupling conditions, thereby modulating the Fano resonance. This strategy is being explored for reconfigurable photonic devices and wearable sensors.
The dynamic control of Fano resonances via external stimuli not only enhances the functionality of plasmonic devices but also opens new avenues for adaptive optics, real-time biosensing, and on-chip optical information processing. Ongoing research at leading institutions and organizations, including Nature Publishing Group and Optica (formerly OSA), continues to advance the understanding and application of tunable Fano resonances in nanophotonics.
Applications in Sensing: Ultra-Sensitive Detection and Biosensing
Fano resonance, characterized by its distinctive asymmetric spectral line shape, has emerged as a powerful phenomenon in the field of plasmonic nanostructures, particularly for applications in ultra-sensitive detection and biosensing. This resonance arises from the interference between a broad spectral continuum and a narrow discrete resonance, leading to sharp features in the optical response of nanostructured materials. In plasmonic systems, such as metallic nanoparticles or nanohole arrays, Fano resonances can be engineered by carefully designing the geometry and arrangement of the nanostructures, enabling precise control over their optical properties.
The unique sensitivity of Fano resonances to changes in the local dielectric environment makes them exceptionally well-suited for sensing applications. When a target analyte binds to the surface of a plasmonic nanostructure, it induces a minute change in the refractive index near the surface. Due to the sharpness of the Fano resonance, even these small perturbations result in significant shifts in the resonance wavelength or intensity, allowing for the detection of extremely low concentrations of biomolecules, pathogens, or chemical species. This property has been harnessed to develop label-free biosensors with detection limits reaching the single-molecule level.
In practical biosensing platforms, Fano-resonant plasmonic nanostructures are often integrated with microfluidic systems or functionalized with specific recognition elements, such as antibodies or aptamers, to achieve selective and real-time detection. The high figure of merit (FOM) associated with Fano resonances—defined as the ratio of sensitivity to the resonance linewidth—enables these sensors to outperform conventional surface plasmon resonance (SPR) sensors in terms of both sensitivity and specificity. This has significant implications for medical diagnostics, environmental monitoring, and food safety, where rapid and accurate detection of trace analytes is critical.
- For example, research supported by organizations such as the National Science Foundation has demonstrated the use of Fano-resonant nanostructures for detecting cancer biomarkers at ultra-low concentrations, paving the way for early disease diagnosis.
- Additionally, collaborative efforts involving the National Institutes of Health have explored the integration of Fano-based plasmonic sensors with portable diagnostic devices, enhancing point-of-care testing capabilities.
The continued advancement of nanofabrication techniques and surface chemistry is expected to further improve the performance and versatility of Fano-resonant plasmonic sensors. As a result, these systems are poised to play a transformative role in next-generation biosensing technologies, offering unprecedented sensitivity, selectivity, and real-time monitoring capabilities.
Fano Resonance in Nonlinear and Active Plasmonic Devices
Fano resonance, a phenomenon characterized by the asymmetric line shape resulting from the interference between a discrete resonance and a broad spectral continuum, has emerged as a pivotal concept in the field of plasmonic nanostructures. In the context of nonlinear and active plasmonic devices, Fano resonances offer unique opportunities for manipulating light-matter interactions at the nanoscale, enabling enhanced optical responses and tunable functionalities.
Plasmonic nanostructures, typically composed of noble metals such as gold and silver, support localized surface plasmon resonances (LSPRs) that confine electromagnetic fields to subwavelength dimensions. When these structures are engineered to support both bright (radiative) and dark (subradiant) modes, their interaction can give rise to Fano resonances. The resulting spectral features are highly sensitive to the geometry, composition, and environment of the nanostructure, making them ideal for applications in sensing, switching, and nonlinear optics.
In nonlinear plasmonic devices, Fano resonances can dramatically enhance nonlinear optical processes such as second-harmonic generation (SHG) and four-wave mixing. The strong local field enhancement associated with the Fano resonance increases the efficiency of these processes, enabling the realization of compact and efficient frequency converters and all-optical switches. Moreover, the sharp spectral features of Fano resonances allow for precise control over the nonlinear response, which is crucial for the development of ultrafast photonic devices.
Active plasmonic devices, which incorporate materials with tunable optical properties (such as semiconductors, phase-change materials, or gain media), benefit from the dynamic control of Fano resonances. By modulating the refractive index or gain in the vicinity of the plasmonic nanostructure, it is possible to actively tune the Fano resonance in real time. This capability is essential for applications in reconfigurable photonic circuits, modulators, and sensors. For instance, integrating graphene or other two-dimensional materials with plasmonic nanostructures enables electrical or optical tuning of the Fano resonance, paving the way for highly responsive and energy-efficient devices.
The study and application of Fano resonances in nonlinear and active plasmonic devices are supported by leading research institutions and organizations such as the Nature Publishing Group, which regularly publishes advances in this field, and the Optica (formerly Optical Society of America), a major professional society dedicated to optics and photonics. These organizations play a crucial role in disseminating knowledge and fostering innovation in the rapidly evolving landscape of plasmonic nanophotonics.
Challenges and Limitations in Real-World Implementations
Fano resonance in plasmonic nanostructures has garnered significant attention due to its potential for highly sensitive optical sensing, enhanced nonlinear effects, and tunable spectral features. However, translating these promising properties from laboratory demonstrations to real-world applications presents several challenges and limitations.
One of the primary challenges lies in the fabrication precision required to achieve and control Fano resonances. The phenomenon arises from the interference between a broad spectral mode (typically a plasmonic resonance) and a narrow discrete mode (such as a localized surface plasmon or a waveguide mode). This interference is highly sensitive to the geometry, size, and arrangement of the nanostructures. Even minor deviations during fabrication—such as edge roughness, size dispersion, or misalignment—can significantly degrade the sharpness and asymmetry of the Fano resonance, reducing device performance. Achieving the necessary nanoscale precision over large areas remains a formidable task, despite advances in techniques like electron-beam lithography and focused ion beam milling.
Another limitation is the material losses inherent to plasmonic metals such as gold and silver. These losses, primarily due to electron scattering and interband transitions, lead to damping of the plasmonic modes and broadening of the resonance features. As a result, the high quality factors (Q-factors) theoretically possible for Fano resonances are often not realized in practice. This limits the sensitivity and selectivity of devices based on these effects. Research into alternative materials, such as doped semiconductors or novel two-dimensional materials, is ongoing but has yet to fully overcome these loss-related challenges.
Environmental stability also poses a significant hurdle. Plasmonic nanostructures are often susceptible to oxidation, surface contamination, and morphological changes under operational conditions, especially when exposed to air, humidity, or biological environments. These factors can alter the local refractive index or the physical structure, leading to unpredictable shifts or degradation of the Fano resonance. Protective coatings and encapsulation strategies are being explored, but they can introduce additional complexity and may affect the optical response.
Finally, scalability and integration with existing photonic and electronic platforms remain challenging. Many Fano-resonant structures are demonstrated on rigid substrates and require complex fabrication steps, making large-scale, cost-effective production difficult. Furthermore, integrating these nanostructures with on-chip systems for practical sensing or signal processing applications requires compatibility with standard manufacturing processes, which is not always straightforward.
Addressing these challenges is a focus of ongoing research, with collaborative efforts from leading scientific organizations such as the Nature Publishing Group and the Optica (formerly Optical Society of America), which regularly publish advances in nanofabrication, materials science, and device engineering relevant to Fano resonance in plasmonic systems.
Future Directions: Emerging Trends and Unexplored Opportunities
Fano resonance in plasmonic nanostructures has emerged as a powerful phenomenon for manipulating light-matter interactions at the nanoscale, offering unique opportunities for sensing, switching, and nonlinear optical applications. As research in this field matures, several future directions and emerging trends are shaping the landscape, while numerous unexplored opportunities remain.
One prominent trend is the integration of Fano-resonant plasmonic nanostructures with active materials, such as phase-change compounds, two-dimensional materials, and quantum emitters. This hybridization enables dynamic control over resonance features, paving the way for tunable optical devices and reconfigurable metasurfaces. For instance, coupling Fano resonances with materials like graphene or transition metal dichalcogenides can yield ultrafast modulators and highly sensitive detectors, leveraging the strong field enhancement and sharp spectral features inherent to Fano systems.
Another emerging direction involves the exploitation of Fano resonances in the mid-infrared and terahertz spectral regions. These frequency ranges are critical for applications in chemical sensing, security screening, and free-space communications. Advances in nanofabrication and material science are enabling the realization of complex plasmonic architectures that support Fano resonances at longer wavelengths, broadening the scope of practical applications.
The development of topologically protected Fano resonances represents a frontier in the field. By designing nanostructures that combine topological photonic concepts with Fano interference, researchers aim to achieve robust, disorder-immune optical responses. Such systems could lead to new classes of sensors and devices with enhanced stability and performance, even in the presence of fabrication imperfections or environmental fluctuations.
Unexplored opportunities also exist in leveraging machine learning and artificial intelligence for the inverse design of Fano-resonant nanostructures. These computational approaches can accelerate the discovery of novel geometries and material combinations that optimize desired optical properties, potentially uncovering unconventional Fano effects and functionalities.
Furthermore, the translation of Fano-resonant plasmonic devices from laboratory demonstrations to scalable, manufacturable technologies remains a significant challenge and opportunity. Collaborations between academic institutions, national laboratories, and industry leaders such as National Institute of Standards and Technology and American Physical Society are expected to play a pivotal role in standardizing fabrication techniques, metrology, and integration strategies.
In summary, the future of Fano resonance in plasmonic nanostructures is marked by interdisciplinary innovation, with promising trends in active hybrid systems, topological photonics, and computational design. As the field advances, it is poised to unlock new functionalities and applications across sensing, communications, and quantum technologies.
Sources & References
- Nature Publishing Group
- Massachusetts Institute of Technology
- Stanford University
- California Institute of Technology
- University of Cambridge
- National Science Foundation
- National Institutes of Health
- National Institute of Standards and Technology